Understanding Constant Energy: A Comprehensive Exploration
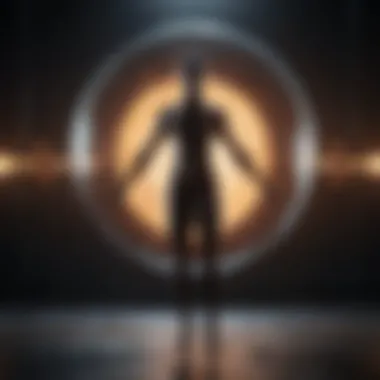
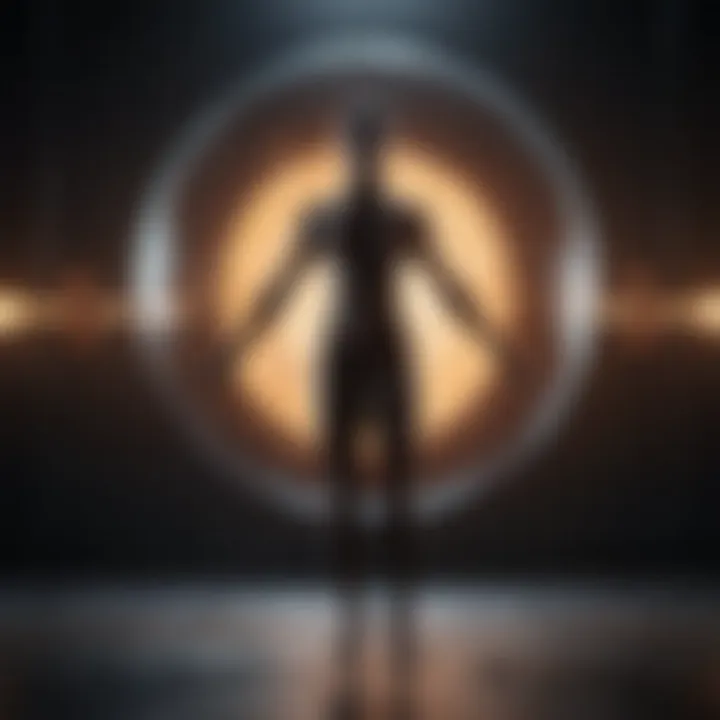
Intro
The concept of constant energy is an essential principle in various scientific disciplines. By studying how energy behaves in different environments, scholars can grasp fundamental laws that govern nature. Constant energy refers to the idea that in a closed system, certain energies remain constant over time. It plays a pivotal role in fields like physics and biology.
Understanding constant energy helps in comprehending complex processes. For instance, in physics, it aids in analyzing systems where energy transformations occur without loss. In biology, it helps scientists explore metabolic pathways. This broad applicability highlights the significance of investigating constant energy.
This article will detail key findings about constant energy, its implications, and future research directions. Researchers and practitioners will benefit from exploring how this topic integrates into current scientific thought.
Preamble to Constant Energy
The concept of constant energy holds a significant place in various scientific discussions, serving as a guiding principle in understanding how energy behaves in physical systems. This importance arises from the need to comprehend the intrinsic properties of energy conservation and its consistent nature across different domains of science. Understanding constant energy has not only theoretical implications but also practical applications in our everyday lives.
By defining constant energy, readers can better appreciate its relevance in various scientific fields, such as physics, biology, and engineering. This understanding is crucial for students, researchers, and professionals who seek to explore the intricate mechanisms that govern energy dynamics. The exploration of constant energy can drive innovation, as it illuminates pathways for developing energy-efficient technologies and methodologies.
Considering constant energy also leads to discussions about energy sources, transfer methods, and the implications of energy conservation principles. This topic becomes even more critical as the world faces challenges related to energy consumption and environmental sustainability.
"Understanding constant energy is not only a theoretical pursuit but a practical necessity in advancing modern science and technology."
Defining Constant Energy
Constant energy refers to a state where the total energy of a system remains unchanged over time. This concept is foundational in scientific principles, emphasizing that energy can neither be created nor destroyed, only transformed from one form to another. In practical terms, this means that in an isolated system, the total energy will stay constant, despite potential changes in form, such as from kinetic to potential energy or thermal energy.
This definition encompasses various forms of energy, including mechanical, thermal, chemical, and nuclear energy. Scientists often utilize this principle to analyze the stability and behavior of systems under various conditions. When one understands the definition of constant energy, it becomes easier to study the effects of energy transformations and to explore energy-efficient systems and technologies that minimize energy loss.
Historical Perspectives on Energy
The understanding of energy and its principles has evolved over centuries. The early philosophical musings on motion and matter set the stage for more scientific inquiries into energy dynamics. Notable figures in the history of physics, such as Galileo Galilei and Isaac Newton, laid crucial groundwork, contributing to the development of principles surrounding motion and force.
The introduction of the first law of thermodynamics in the 19th century marked a significant milestone. This law formalized the concept of energy conservation, stating that the total energy in an isolated system remains constant. The implications of this law extended beyond physics into other fields such as chemistry and biology, illuminating the universal nature of energy conservation.
Further advancements, particularly during the industrial revolution, showcased the practical applications of energy principles. As society focused on harnessing energy for various technologies, an understanding of constant energy became essential. Developing efficient engines and energy systems relied heavily on a comprehensive understanding of energy conservation and transformation processes.
The Principles of Energy Conservation
The principles of energy conservation form a foundational aspect of our understanding of constant energy. Established laws dictate that energy cannot be created or destroyed, only transformed from one form to another. This law is integral to many scientific fields, influencing theories and applications in physics, engineering, and biology. By adhering to these principles, scientists and engineers can design systems that optimize energy use, contributing to sustainable practices.
In practical terms, the importance of these principles lies in several key elements. They elucidate the behavior of energy in various contexts, enabling us to predict outcomes and understand interconnected systems. Recognizing how energy flows and transforms provides clarity on efficiency, ultimately guiding design choices in technology and conservation efforts.
First Law of Thermodynamics
The first law of thermodynamics is a cornerstone of energy conservation. It states that the total energy in a closed system remains constant; thus, energy can neither be created nor destroyed. This principle is paramount in thermodynamic processes, illustrating how heat is converted to work and vice versa. In essence, the energy before a process must equal the energy after.
Practical examples can be found in mechanical systems where potential energy converts into kinetic energy during motion or in thermal systems where heat exchange occurs. Engineers apply this principle to ensure energy efficiency in engines and other systems, making design choices that minimize energy loss.
Energy Transfer Mechanisms
Energy transfer mechanisms explain how energy moves from one system to another. These mechanisms include conduction, convection, and radiation, which are key to understanding heat transfer.
- Conduction occurs when energy is transferred through direct contact between materials. For example, touching a hot stove transmits heat to your hand.
- Convection involves the movement of fluids, in which warmer areas of liquid or gas rise, while cooler areas sink, creating a cycle of heat exchange.
- Radiation allows energy to transfer through electromagnetic waves, like sunlight warming the Earth.
Each transfer mechanism plays a crucial role in many applications, such as heating systems or weather phenomena. By understanding these mechanisms, researchers can improve energy usage, design better thermal insulation, and develop efficient heating and cooling solutions.
"Energy transfer mechanisms are essential for efficiency in applications and fundamental to the study of thermodynamics."
Appreciating these principles and mechanisms allows for more informed decisions in technology and sustainable practices.
Mathematical Formulations of Constant Energy
The mathematical formulations of constant energy are essential in understanding both theoretical and practical applications across various fields. They serve as the backbone for analyzing physical systems where energy remains unchanged. Mastery of these formulations enables scientists and engineers to predict system behaviors, optimize performance, and troubleshoot issues that may arise in different contexts. Therefore, delving into equations and theorems related to constant energy equips researchers with critical tools for innovation.
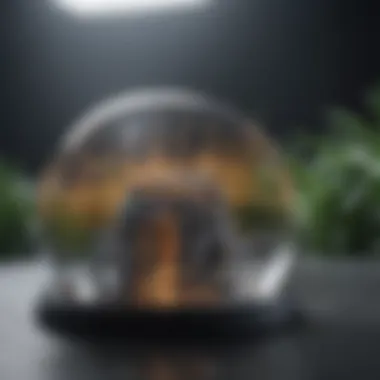
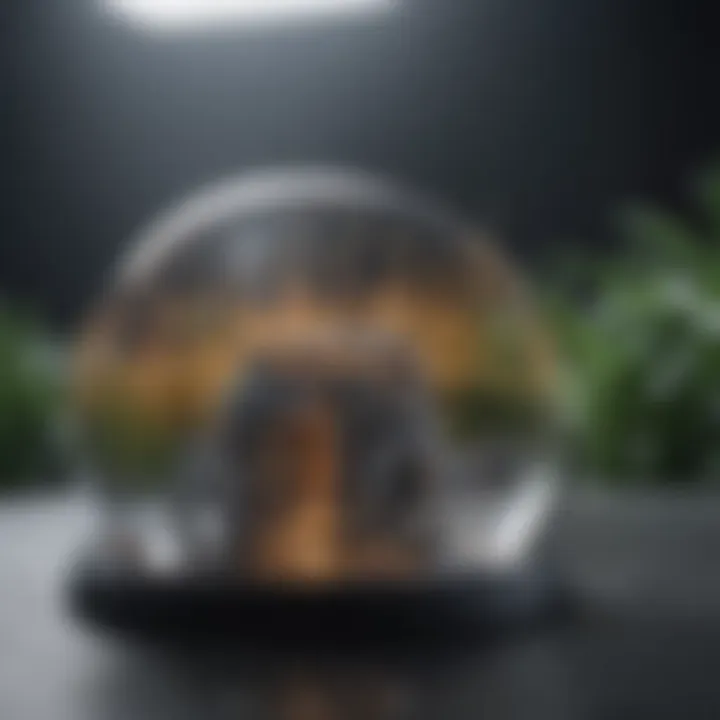
Equations and Theorems
At the foundation of constant energy are key equations that describe how energy behaves under constant conditions. The most notable is the conservation of energy equation, which states that the total energy within a closed system remains constant over time. Mathematically, it can be represented as:
[ E_total = KE + PE = constant ]
Where ( KE ) denotes kinetic energy and ( PE ) represents potential energy.
Another significant theorem includes the work-energy principle, articulating that the work done on a system is equal to the change in its kinetic energy. This can be expressed as:
[ W = \Delta KE = KE_final - KE_initial ]
These formulations highlight the interplay between energy types and their transformation rather than destruction. Furthermore, they provide foundational support for more complex analyses, such as those found in thermodynamics.
Application of Calculus
Calculus plays a crucial role in the mathematical formulations of constant energy. It helps in formulating energy-related insights through differential equations. For instance, in situations where energy relates to motion, calculus enables the derivation of formulas to ascertain velocity and acceleration over time.
The application of integral calculus is also vital when determining the work done by a force over a distance. This can be expressed as:
[ W = \int F dx ]
Where ( F ) is the force applied and ( x ) is the distance moved.
These mathematical tools not only allow for practical applications in engineering but also support deeper understandings in physics, particularly in identifying how energy switches from one form to another without loss or gain, thereby affirming its constant nature.
"The laws of thermodynamics provide a comprehensive framework for understanding how energy behaves in all forms."
By utilizing these equations and principles, it is possible to create simulations and models that predict outcomes in real-world energy systems. The symbiosis between mathematical formulations and practical applications signifies the power of mathematics in comprehending constant energy.
Constant Energy in Classical Mechanics
In the realms of classical mechanics, the concept of constant energy serves as a backbone for understanding movement and forces. This section delves into the intricate details of kinetic and potential energy, as well as the work-energy theorem, which collectively outline how energy is conserved in dynamic systems. Recognizing the significance of these principles is vital for students and researchers in comprehending both the fundamental theories and practical applications that stem from classical mechanics.
Kinetic and Potential Energy Dynamics
Kinetic energy refers to the energy an object possesses due to its motion. It is defined by the equation:
[ KE = \frac12 mv^2 ]
where m represents mass and v indicates velocity. As an object accelerates, its kinetic energy increases, showcasing a direct relationship between mass, velocity, and energy. This relationship is crucial in many physics applications, from simple projectile motions to complex machinery.
Potential energy, on the other hand, embodies energy stored in an object due to its position or configuration. A common example is gravitational potential energy, expressed as:
[ PE = mgh ]
where h denotes height above a reference point and g is the acceleration due to gravity. The interplay between kinetic and potential energy illustrates the principle of energy conservation, where the total mechanical energy of a closed system remains constant in the absence of external forces.
In classical mechanics, these energy dynamics provide a framework for analyzing systems, whether debating the trajectory of a falling object or examining the oscillations of a pendulum. The exchange between kinetic and potential energy reveals how forces act and maintain balance within various systems.
Work-Energy Theorem
The work-energy theorem posits that the work done on an object is equal to the change in its kinetic energy. This principle can be summarized as follows:
[ W = \Delta KE = KE_final - KE_initial ]
Here, W signifies work, which is the product of force and displacement. This theorem is fundamental in classical mechanics as it links force, energy, and motion, offering critical insights in mechanical analysis.
The implications of the work-energy theorem extend to different scenarios, including variable forces and conservation of energy in isolated systems. When a force acts over a distance, energy is transferred, either increasing or decreasing the kinetic energy of an object. In practical terms, understanding work and energy allows for better insights into systems such as engines, roller coasters, and various mechanical devices.

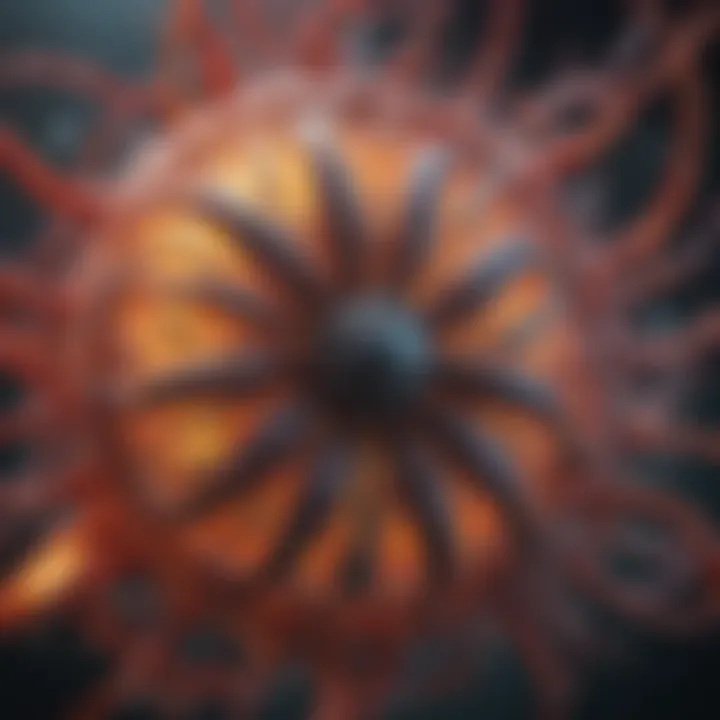
To sum it up, the principles of kinetic and potential energy, along with the work-energy theorem, provide a comprehensive framework for evaluating classical mechanics. Their significance is not just theoretical; they apply to various scientific and engineering problems, influencing both the academic study and practical application of energy across numerous fields.
Constant Energy in Quantum Physics
Constant energy serves as a pivotal concept in quantum physics, underpinning the behavior and interactions of particles at the quantum level. This section discusses how the principles of constant energy shape our understanding of quantum states and the implications these have for the broader scientific community.
Quantum States and Energy Levels
Quantum states represent the conditions at which particles exist within quantum mechanics. Each state has a specific energy level associated with it, and the principle of constant energy plays a critical role in these configurations. For instance, electrons in an atom reside in distinct energy levels, with the possibility of transitions between them. These transitions occur when an electron absorbs or emits energy, but the overall system maintains constant energy when considering the entire ensemble.
The quantized nature of energy levels in quantum systems means that particles cannot possess arbitrary energy values. Instead, they are limited to specific amounts, resulting in unique phenomena such as spectral lines and quantum jumps. The implications here are profound, as they allow for the prediction of chemical reactions and the design of various quantum technologies.
The Role of Constant Energy in Quantum Mechanics
In quantum mechanics, constant energy refers to the notion that the total energy of an isolated system remains unchanged over time. This principle is foundational to various equations, such as the Schrödinger equation, which governs how quantum states evolve.
Moreover, the principle of conservation of energy in quantum contexts leads to significant advancements in technology. For example, lasers and semiconductors operate based on the delicate balance of energy levels and transitions, benefitting from the predictability allowed by constant energy. Such technologies have revolutionized fields like electronics and communications, enhancing our society significantly.
"The conservation of energy in quantum mechanics informs not only theoretical frameworks but also practical applications ranging from basic research to advanced technology development."
In summary, constant energy in quantum physics not only elucidates the essential behavior of quantum particles but also provides a framework for innovations that drive contemporary advancement. Understanding these interactions is crucial for both theoretical physicists and engineers working at the nexus of physics and technology.
The Role of Constant Energy in Biology
Constant energy plays a crucial role in biology, impacting various processes integral to life. Understanding how constant energy functions in biological systems helps elucidate fundamental mechanisms underlying growth, reproduction, and metabolism. By examining energy transfer within biological frameworks, we gain insights into how organisms maintain homeostasis and adapt to their environments.
Metabolism and Energy Conservation
Metabolism encompasses the complex biochemical reactions that occur within living organisms. It includes catabolic processes, which break down molecules to release energy, and anabolic processes, which use energy to construct cellular components. The concept of constant energy is essential in understanding how organisms effectively manage these biochemical reactions.
Energy conservation within metabolism works primarily through ATP, or adenosine triphosphate. ATP acts as an energy currency within cells, allowing for energy to be stored and utilized efficiently. Organisms must continuously convert one form of energy into another, whether it is from sunlight in plants or nutrients in animals. Factors influencing metabolism include:
- Enzyme activity
- Temperature
- Nutrient availability
The ability of an organism to sustain its energy levels directly affects its metabolism, growth, and overall health. In essence, the balance between energy input and output determines metabolic efficiency.
Biological Implications of Energy Transfer
Energy transfer in biological systems has significant implications for both individual organisms and ecosystems. The way energy is utilized affects population dynamics, biodiversity, and evolutionary processes. When energy is converted, it often involves losses due to entropy, which is a natural aspect of energy transformations.
"Energy transfer in ecosystems defines how species interact, evolve, and survive."
Understanding energy transfer can lead to improved conservation strategies. For example:
- Fossil fuel reliance contributes to energy depletion and environmental damage.
- Sustainable practices in agriculture promote energy efficiency.
- Ecosystem preservation ensures energy flow remains intact.
Engineering Applications of Constant Energy
The exploration of constant energy finds significant relevance within the field of engineering. As engineers strive to create efficient systems that maintain energy levels, understanding the principles of constant energy aids in optimizing device performance and sustainability. Recognizing the interplay between energy inputs and outputs directly influences design decisions, ensuring that energy is conserved and utilized effectively across various applications.
Energy Systems in Engineering
The foundation of energy systems in engineering lies in the principles of constant energy. These systems are designed to maximize utility while minimizing waste. Various types of energy systems include power plants, renewable energy systems, and energy storage solutions. In many cases, the goal is to maintain a constant energy output, which requires a delicate balance of inputs.
- Power Plants: Conventional power plants work on the fundamentals of constant energy to regulate energy production. The efficiency of these installations directly impacts the overall energy grid.
- Renewable Energy Systems: Solar panels and wind turbines must harness energy consistently. Engineers design these systems to not only capture renewable energy but also convert and store it effectively.
- Energy Storage Solutions: Technologies such as batteries and fuel cells are engineered to maintain constant energy output over time. Their designs focus on maximizing energy retention and minimizing loss.
Managing the complexities of these systems requires a strong understanding of thermodynamics and energy transfer principles. Engineers must consider factors such as thermal efficiency, energy density, and load balancing. High demand periods necessitate quick adaptive responses to maintain energy levels while optimizing resource use.
Design Principles for Energy Efficiency
Incorporating constant energy concepts into design principles promotes enhanced energy efficiency. Various strategies are essential for engineers to integrate into their projects.
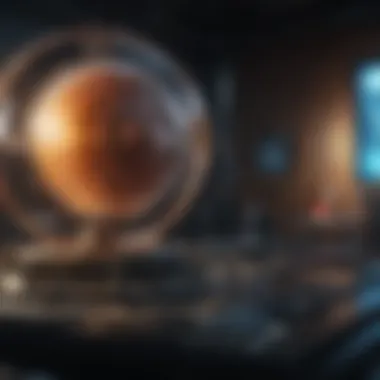
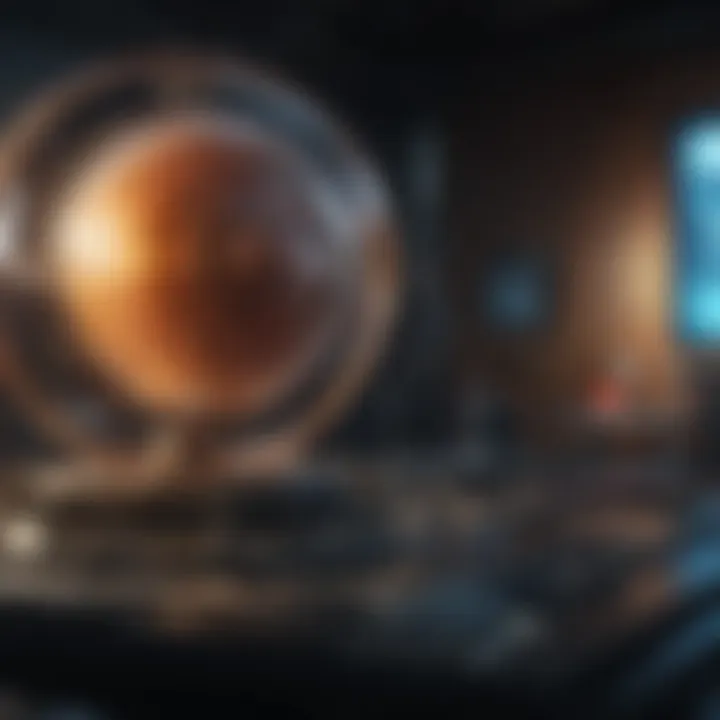
- Material Selection: Choosing materials with better thermal properties minimizes energy loss. For instance, insulating materials are crucial in buildings to maintain desired temperatures with lower energy consumption.
- Control Systems: Advanced control systems can automatically adjust energy output based on real-time demand. These systems utilize sensors and feedback mechanisms to regulate energy use dynamically.
- Sustainable Practices: Employing sustainable design practices aids in reducing energy demand, such as incorporating energy-efficient technologies and renewable energy sources.
- Lifecycle Analysis: Evaluating the entire lifecycle of a product, from production to disposal, helps engineers identify energy consumption hotspots, allowing for targeted improvements in energy efficiency.
"Engineering’s role in constant energy extends beyond merely solving problems; it emphasizes the necessity of innovation and foresight in the sustainable development of systems that support modern life."
Ultimately, the engineering applications of constant energy are pivotal in addressing contemporary challenges related to energy efficiency and sustainability. By implementing thoughtful design and operational strategies, the field advances towards a future that minimizes waste while maximizing utility.
Challenges in Achieving Constant Energy Systems
The pursuit of constant energy systems in various scientific and engineering contexts faces numerous challenges. Understanding these challenges is crucial for anyone engaged in related research or practical applications. Exploring the obstacles can inform future advancements and inspire innovative approaches in energy systems.
Entropy and Energy Dissipation
Entropy plays a significant role in the challenges faced within constant energy systems. It is a measure of disorder in a system and, according to the second law of thermodynamics, entropy tends to increase over time. This increase in entropy implies that systems cannot attain absolute efficiency in energy usage. As energy is transferred or transformed, some of it inevitably dissipates as waste heat, which reduces the overall energy available for work. This is critically important in contexts such as mechanical systems, where friction leads to energy losses.
In practical terms, designing systems that can minimize energy dissipation is challenging. Engineers must find ways to create materials and mechanisms that reduce friction and thermal losses. While technologies such as superconductors can enhance energy efficiency, they require specific conditions and may not be practical for all applications. Hence, addressing entropy and energy dissipation is essential for achieving more effective constant energy systems.
Limitations in Practical Applications
When discussing constant energy systems, limitations often become apparent in practical applications. First, many theoretical models do not translate well into real-world scenarios. For example, ideal conditions assumed in mathematical derivations may not exist in practical systems. This discrepancy highlights the complexity of real-world variables like unpredictable environmental factors and material limitations.
Moreover, economic factors cannot be ignored. Developing constant energy systems often requires significant initial investment in technology and infrastructure. Potential returns on investment may take years to materialize, leading many organizations to hesitate in making commitments.
In addition, regulatory and safety concerns can restrict the implementation of certain technologies. For instance, while renewable energy sources present promising prospects, their efficient integration into existing grids and systems often faces bureaucratic hurdles.
The Future of Constant Energy Research
The exploration of constant energy is not merely a scientific endeavor; it represents an essential pillar in the development of future technology. The increasing awareness of energy sustainability and the pressing need to optimize energy consumption have put constant energy systems under a critical lens. Researchers and practitioners in fields such as physics, engineering, and environmental science are particularly focused on how these constant energy principles can be applied to solve real-world problems. As the demand for efficient energy systems grows, so does the interest in exploring new methodologies and technologies that adhere to the principles of constant energy conservation.
Emerging Technologies and Innovations
In recent years, numerous technologies have emerged that harness the concept of constant energy. These innovations have vast implications in various sectors, including renewable energy, electronics, and transportation.
- Renewable Energy Systems: Advancements in solar panel efficiency and wind energy conversion have been prominent. Technologies like concentrated solar power systems and advanced vertical-axis wind turbines are becoming more efficient and effective.
- Energy Storage Solutions: Innovations in battery technology, such as lithium-sulfur and solid-state batteries, are providing new ways to store energy more effectively. These storage systems help ensure that the energy generated from renewable sources can be utilized when needed, maintaining a balance in energy supply and demand.
- Smart Grids: The development of smart grid technology integrates constant energy concepts to enhance energy distribution and reduce waste. This manages energy flow efficiently, ensuring that supply meets demand in real-time.
The integration of these technologies signifies a major leap towards achieving practical applications of constant energy systems, making them highly relevant for current and future research initiatives.
Sustainability and Energy Solutions
Constant energy research is also inherently tied to discussions on sustainability. The commitment to reducing carbon footprints and enhancing energy efficiency drives the exploration of sustainable energy solutions.
- Impact on Climate Change: Research into constant energy can help allocate resources more efficiently, thereby reducing greenhouse gas emissions. This is particularly critical as societies seek alternatives to fossil fuels.
- Resource Management: The concepts of constant energy provide frameworks for managing energy resources more wisely, ensuring that future generations have access to necessary energy sources.
- Enhancing Energy Accessibility: The future of constant energy research holds promise for developing solutions that make energy more accessible to underserved populations. Through innovations in energy systems, it is possible to provide affordable and sustainable energy to those who need it most.
As we look ahead, the pursuit of constant energy systems will undoubtedly lead to crucial advancements in both technology and sustainability initiatives. The efforts of researchers, combined with technological innovations, culminate in a comprehensive approach to mitigating the energy challenges faced by our society.
"Sustainable energy solutions are no longer merely an option; they are an imperative for the future."
Emphasizing the importance of constant energy research will not only influence future innovations but also play a fundamental role in reshaping how we understand and interact with energy systems globally.
Epilogue
The conclusion of this article encapsulates the essential themes surrounding constant energy. In evaluating the diverse realms in which constant energy has significant implications, one recognizes its profound role within scientific exploration, technology, and biological systems. Understanding constant energy fosters an appreciation for how energy conservation principles intersect with practical applications and theoretical frameworks.
Summary of Key Insights
Throughout the article, key insights emerge regarding the definition and significance of constant energy. Some of the most crucial points include:
- Interdisciplinary Relevance: Constant energy is pivotal across disciplines such as physics, biology, and engineering, highlighting its universal applicability.
- Energy Conservation Principles: The discussion encompasses fundamental conservation laws, emphasizing their role in understanding energy dynamics in various systems.
- Real-World Applications: Practical examples illustrate how theories translate into technologies aimed at enhancing energy efficiency and sustainability, revealing the direct connection between theory and practice.
These insights are not only theoretical but also possess a tangible impact on the way society approaches energy-related challenges. By prioritizing constant energy studies, future innovations can arise that forge paths for enhanced efficiency and sustainability in various fields.
Implications for Future Research
The implications for future research in the realm of constant energy are multiple and pressing. Several areas warrant particular attention:
- Advancements in Energy Efficiency: Investigating newer systems that better embody the principles of constant energy can potentially yield methods that minimize waste and increase productivity in energy usage.
- Sustainability Research: Exploring the application of constant energy in renewable technologies may illuminate paths towards sustainable practices that benefit both science and society.
- High-Energy Physics Investigations: In-depth studies into quantum states and energy levels could provide further insights into energy quantization. This may impact how we perceive energy within the universe.
As the landscape of energy research continues to evolve, staying attuned to the advancements of constant energy will become increasingly vital. The convergence of technology with the principles of constant energy holds promise for a future where both research and application coexist harmoniously.