Molecular Biology of Plants: Mechanisms and Interactions
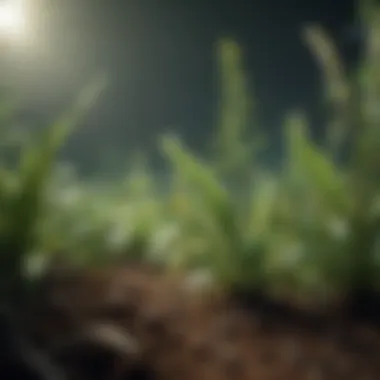
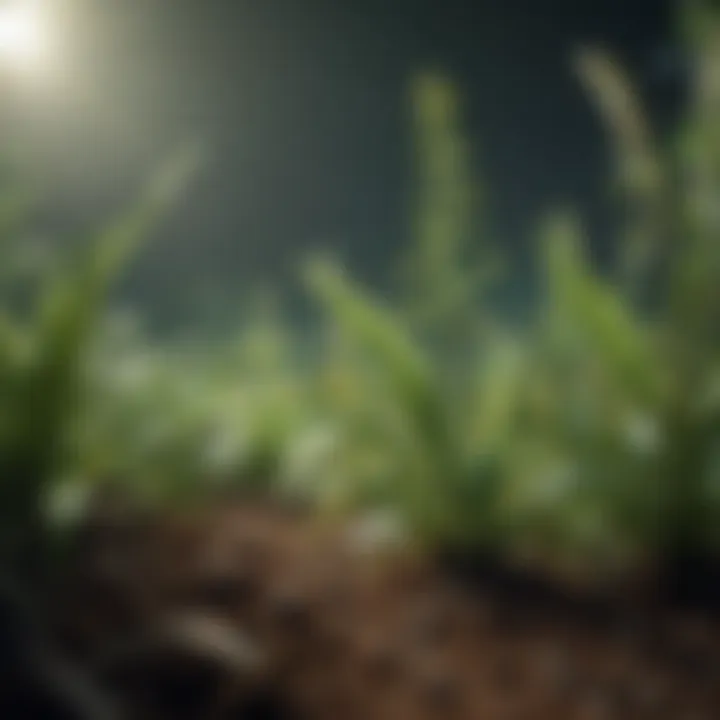
Intro
Plants play a fundamental role in our ecosystem, acting as the backbone of life on Earth. Understanding their molecular biology uncovers how they adapt, grow, and thrive under varying environmental conditions. At a molecular level, plants are complex organisms with intricate networks of interactions that guide their development and responses to stimuli. This exploration illuminates critical biological processes, emphasizing how genetic expressions and signaling pathways orchestrate plant life.
Genetic variation is key in shaping plant responses. Factors such as soil quality, sunlight, and water availability all play a role in determining how genes express themselves, leading to observable traits. As we dive deeper, it's essential to consider how these processes can lead to innovations in agriculture, making strides in crop sustainability and productivity.
Key Findings
- Summary of the main results
The molecular mechanisms that drive plant growth and adaptivity are vast and multi-faceted. Research has shown that specific genes are activated in respond to environmental stressors, enabling plants to allocate resources where they are most needed. For example, drought-tolerant plants have unique gene expressions that allow them to conserve water, showcasing nature's resilience. - Significance of findings within the scientific community
Findings related to plant molecular biology hold significant value among researchers. With climate change posing unprecedented challenges, understanding how plants cope with stress not only contributes to botanical knowledge but is also essential for developing strategies to enhance agricultural productivity in a changing environment.
Implications of the Research
- Applications of findings in real-world scenarios
The insights derived from molecular biology of plants can reshape agricultural practices. Farmers can adopt new technologies informed by genetic research to implement crop varieties that are more resilient to extreme weather. Biotechnical advances allow us to engineer plants with improved traits, thereby ensuring food security. - Potential impact on future research directions
As we gather more data on genetic pathways and signaling processes, we can expect future research to focus on genomic editing techniques, like CRISPR, enhancing our ability to fine-tune crop traits. The implications stretch from improving nutritional content to increasing yield in varying climates, a promising frontier for food sustainability.
"Understanding plant biology isn't just academic; it's the key to ensuring our food systems can withstand the challenges of tomorrow."
It's clear that the relationship between molecular biology and botanical adaptation is a complex and sophisticated dance. This article serves as a starting point for delving into the remarkable world of plant science, encouraging a deeper appreciation for the natural processes that enable life to flourish.
Foreword to Plant Molecular Biology
Molecular biology of plants sits at the fascinating intersection of biology and agriculture, playing a pivotal role in understanding how plants function at a molecular level. This burgeoning field is crucial because it unlocks the complex mechanics behind everything from plant growth and development to resilience against environmental stresses. By grasping these detailed processes, researchers, educators, and professionals can innovate agricultural practices, enhance crop yields, and contribute to global food security.
In this article, weâll explore several key aspects of plant molecular biology. We will dive into the genomic structure, gene expression regulation, molecular mechanisms of development, and other essential elements. Understanding these facets will help illuminate the pathways through which plants respond to their surroundings and adapt to changing environments.
Definition and Importance
Plant molecular biology refers to the study of the molecular mechanisms that govern plant structure and function. This includes the investigation of genes, their expression, and how they interact with one another and with environmental factors to influence plant behavior. The importance of this field cannot be overstated, as it not only sheds light on basic biological questions but also has profound implications for agriculture and environmental sustainability.
- Enhanced Crop Resilience: As we face climate change, understanding molecular responses can help us breed plants that withstand drought, salinity, and disease.
- Biotechnological Innovations: Techniques such as genetic engineering and CRISPR can be applied to improve crop traits, leading to higher yields or nutritional content.
- Ecological Balance: Knowledge garnered through plant molecular biology can assist in ensuring that agricultural practices do not upset local ecosystems.
This definition sets the stage for exploring how different molecular mechanisms intertwine within plant systems.
Historical Context
The roots of plant molecular biology trace back to the early 20th century, when scientists first began to study heredity in plants. Gregor Mendelâs pea plant experiments laid the groundwork for our understanding of genetics. This was further developed through breakthroughs such as the discovery of DNA's structure by Watson and Crick in the 1950s, which made genetics a focal point in biological research.
With the advent of molecular techniques in the 1970s, like recombinant DNA technology, research began diving deeper into specific plant genes and their functions. For instance, the isolation of the first plant gene, known as the Chlorophyll a/b-Binding Protein gene, was a significant milestone achieved in the 1980s. This paved the way for more recent advancements such as genomics and transcriptomics, bringing us to where we are today.
In the modern era, large-scale sequencing projects and bioinformatics tools have revolutionized plant molecular biology, enabling us to annotate entire plant genomes and measure gene expression in real-time.
Understanding this historical context is essential for appreciating the advancements that have led to the current scope of plant molecular biology. This growing discipline continues to evolve, promising even more innovative contributions to both science and agriculture.
Genomic Structure of Plants
Understanding the genomic structure of plants is akin to deciphering the blueprint of life itself. Plant genomes carry critical instructions that inform various responses to environmental stimuli, development patterns, and even the overall fitness of the plant. By analyzing these genomic structures, researchers can unlock the secrets of plant adaptability, productivity, and resilience in changing climates. This section digs deep into the configurations that make plants unique and why this knowledge is essential for both academic pursuits and practical applications.
Understanding Plant Genomes
Plant genomes typically exhibit remarkable complexity, with variations in size, structure, and gene content across species. One of the most significant aspects of plant genomes is polyploidy, where plants have multiple sets of chromosomes. For instance, wheat is hexaploid, carrying six sets of chromosomes. This trait can confer advantages, such as increased robustness and the ability to adapt to diverse environments. The human utilization of these traits has spurred various agricultural advancements, impacting food security on a global scale.
Genomic studies help us identify functional genes vital for plant traits. Techniques like whole-genome sequencing allow researchers to map genomes in detail. For example, in the case of Arabidopsis thaliana, a model organism in plant studies, sequencing its genome has opened new avenues to studying genetic functions and their impacts on growth mechanisms. Furthermore, the understanding of non-coding DNA regionsâonce termed as "junk" DNAâhas evolved. These regions can regulate gene expression, influencing plant development and responses.
Plant genomes not only encode information for growth, but they also reveal how plants survived through millennia of evolution.
Comparative Genomics
Comparative genomics studies the similarities and differences in genomes across various plant species, and it holds great promise for unraveling the intricate network of plant traits and divergences.
By examining genomes from a variety of plants, scientists can identify conserved genes that play crucial roles across different species. For example, researchers may compare the genomes of maize and sorghum. Despite their distinct phenotypes and cultivation requirements, certain genes related to stress tolerance may be remarkably similar. This information is invaluable for breeding programs aimed at enhancing crop resilience.
This comparison can also shed light on evolutionary processes. Different adaptation strategies, often influenced by environmental pressures, become apparent through genomic insights. For instance, utilizing information from wild relatives can prove essential when tackling challenges like diseases and climate stressors.
In summary, the genomic structure of plants not only provides insight into their biological functions but also lays the groundwork for future innovations in plant science and agriculture. Through careful dissection of plant genomes and their comparison, researchers can make strides in cultivation techniques, ultimately addressing global food demands and environmental challenges.
Gene Expression Regulation
Gene expression regulation is a cornerstone of molecular biology that governs how genetic information flows from DNA to RNA to proteins in plants. This process is vital for a variety of reasons. First off, it ensures that the right genes are expressed at the right time, allowing plants to adapt to various environmental conditions. Without such regulation, plants would struggle to thrive, unable to respond appropriately to changes such as light availability, water supply, and nutrient levels.
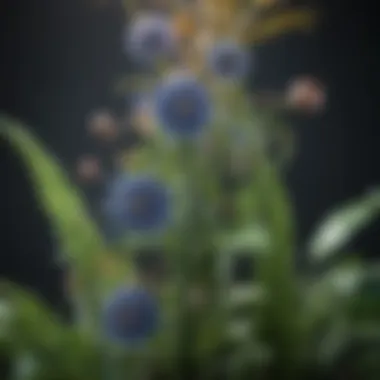
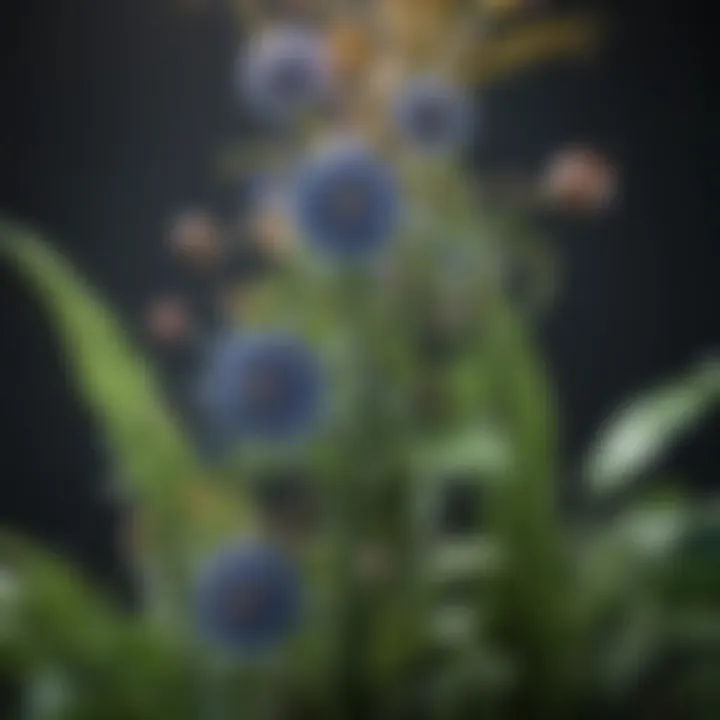
Moreover, the concept of gene expression regulation intersects beautifully with many facets of plant biology including development, metabolism, and stress responses. For example, when a plant undergoes drought stress, specific genes responsible for water conservation can be upregulated, while others might be turned down. Thus, understanding how gene expression regulation works is not just academic; it has real-world implications for crop resilience and food security.
Transcription Factors and Their Roles
Transcription factors (TFs) serve as the conductors in the vast symphony of gene expression. These proteins interact with specific DNA sequences, facilitating or hindering the transcription of target genes. It's worth noting that TFs can act as activators, enhancing gene expression, or repressors, when they inhibit it. For instance, when plants experience stress, certain TFs respond swiftly, initiating a cascade that may result in the activation of genes that help in stress mitigation.
Some prominent examples of transcription factors in plants include MYB, NF-Y, and AP2/ERF families. Each of these families regulates specific aspects of plant growth and development, such as flowering time, fruit ripening, or leaf senescence. Understanding these roles helps researchers develop strategies to improve plants, making them more resilient to climate uncertainties.
- MYB: Known for its role in regulating the biosynthesis of flavonoids and anthocyanins, crucial for flower pigmentation.
- NF-Y: Plays a key role in the flowering process by regulating genes involved in flowering time.
- AP2/ERF: Particularly important in mediating response to abiotic stresses, such as drought and salinity.
But itâs not just about the function of individual transcription factors; their interactions within networks paint a more complex picture. These networks ensure that plants fine-tune their responses to environmental cues. So the next time you admire a blooming flower, remember the intricate dance of transcription factors that brought it to life.
Post-Transcriptional Modifications
Once the RNA is transcribed, it doesn't always imply that it will be translated into a protein right away. Post-transcriptional modifications play a significant role in regulating gene expression after transcription. These modifications, which include splicing, capping, and polyadenylation, affect RNA stability and translation efficiency.
- Splicing: This process involves the excision of introns and the joining of exons. Alternative splicing contributes to the diversity of proteins, which is essential for plants to respond dynamically to their environment.
- Capping: The addition of a 5â cap to the RNA helps in stabilization and plays a crucial role in the initiation of translation.
- Polyadenylation: The addition of a poly(A) tail to the 3â end of RNA enhances its stability and is crucial for the process of translation.
These modifications do not just add a layer of complexity but also offer plants additional means to adapt and interact with their environments. For example, many plants have adapted mechanisms to change splicing patterns in response to stresses, giving them an edge in survival.
âGene expression is a dynamic system; understanding it is crucial not only for biological insights but also for agricultural advancements.â
Molecular Signaling Pathways
Molecular signaling pathways form the backbone of plant biology, acting as the conduits for transmitting information within plant cells and between them. These pathways are not only crucial for growth and development but also play a major role in how plants interact with their environment. Without these sophisticated systems, plants would struggle to adapt to changing conditions, limiting their survival. The significance of these pathways lies in their ability to integrate various stimuli, enabling plants to make life-sustaining decisions such as when to grow, flower, or initiate defense mechanisms against pests.
Hormonal Regulation
Hormonal regulation is one of the most imperative aspects of molecular signaling pathways. Different hormones play unique roles in plant development, influencing everything from growth direction to stress responses. Within this category, five key hormones stand out:
Auxins
Auxins are vital hormones responsible for promoting cell elongation, thus playing a central role in the plant's growth patterns. A characteristic trait of auxins is their ability to facilitate phototropism, where plants grow towards light. This makes auxins crucial for optimizing photosynthesis, allowing the plant to harness energy effectively. A unique feature of auxins is their varying concentration across different plant tissues, which enables targeted growth responses. However, excessive auxin levels can lead to abnormal growth, illustrating the need for balanced regulation in practical applications.
Gibberellins
Gibberellins are another influential group of hormones that support stem elongation and seed germination. Known for their distinct ability to break dormancy, gibberellins act as a signaling molecule that triggers seed growth when environmental conditions are favorable. This characteristic makes them particularly beneficial for agricultural practices focused on improving crop yield. The unique aspect of gibberellins lies in their synthetic formulations, which can be employed to accelerate maturity in crops. A downside to consider is that improper application can lead to overgrowth or weaker plants, highlighting the need for careful management.
Cytokinins
Cytokinins support cell division and differentiation, acting as key players in plant tissue culture and organogenesis. Their primary characteristic is the ability to promote lateral shoot growth, which can result in increased yield potential. What's interesting about cytokinins is that they counteract the effects of auxins, balancing growth processes. Their role in delaying leaf senescence is another advantage, enhancing the photosynthetic activity of older leaves. On the flip side, too much cytokinin can lead to uncontrolled proliferation, necessitating cautious regulation in biotechnological applications.
Ethylene
Ethylene is a gaseous hormone known for its roles in fruit ripening and flower wilting. An important characteristic of ethylene is its ability to regulate processes such as abscissionâwhere leaves or fruit detach from a plant. This makes ethylene crucial for agricultural practices, such as inducing uniform ripening of fruit. A unique feature of ethylene is its feedback mechanism; increased levels of ethylene can stimulate further production, creating a cascading effect. However, this can also be a double-edged sword, as uncontrolled ethylene production can lead to premature aging of plant tissues.
Abscisic Acid
Abscisic acid is most recognized for its role in plant stress responses, particularly during droughts. The primary characteristic of this hormone is that it promotes stomatal closure, reducing water loss during adverse conditions. This makes it a particularly valuable subject of study for improving plant resilience in increasingly harsh climates. A unique aspect of abscisic acid is its ability to signal other stress-related pathways, making it a multifaceted player in plant defense. Still, its involvement in growth inhibition can present challenges, particularly in agriculture where a balance between growth and stress response is necessary.
Stress Response Mechanisms
Stress responses in plants encompass a range of complex signaling pathways that activate upon environmental challenges, such as drought, salinity, or pathogen attacks. These mechanisms serve as the plant's first line of defense, enabling rapid adaptations to survive external pressures. The importance of comprehending these pathways extends beyond basic biology; they hold significant implications for improving crop resilience and stability in the face of climate change and evolving agricultural demands. This necessity drives ongoing research into elucidating the intricate web of interactions among various signaling pathways and their roles in plant survival.
Molecular Mechanisms Underpinning Development
The realm of plant molecular biology is deeply intertwined with how plants develop from a mere seed into a robust structure full of life. Focusing on the molecular mechanisms underlying development offers us critical insights into how plants differentiate cells and respond to their environment. This understanding is paramount, especially in the context of agriculture and biodiversity, where enhancing growth patterns and resilience can lead to sustainable food sources.
Cell Differentiation and Development
In the life cycle of a plant, cell differentiation plays a key role. This process determines how generic plant cells become specialized for certain functions. For instance, some cells will become part of the leaf, while others evolve into roots or flowers. Every layer of complexity in plant structures stems from this process, illustrating the sophisticated machinery at work in plant biology.
Several factors drive cell differentiation, including genetic factors and environmental stimuli. One prominent example arises from the signaling molecules known as auxins. These hormones orchestrate the growth and establishment of various plant structures, acting like conductors in an orchestra of cellular activity.
A deeper look reveals how gene expression is fundamentally linked to differentiation. Different cell types express distinct sets of genes, which in turn regulate their unique functions. For instance, root cells develop characteristics that allow them to absorb water and nutrients efficiently, while leaf cells take on properties that enable photosynthesis. The specificity of gene expression is underpinned by a network of transcription factors that bind to DNA and activate or repress target genes based on developmental cues and environmental conditions.
Moreover, epigenetic modifications also play a pivotal role in cell differentiation by altering how genes are expressed without changing the underlying DNA sequence. These modifications can have lasting effects, contributing to phenotypic variation that allows plants to adapt to changing environments.
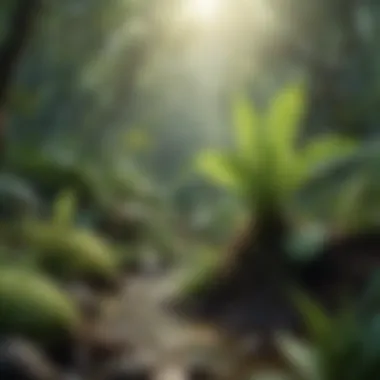
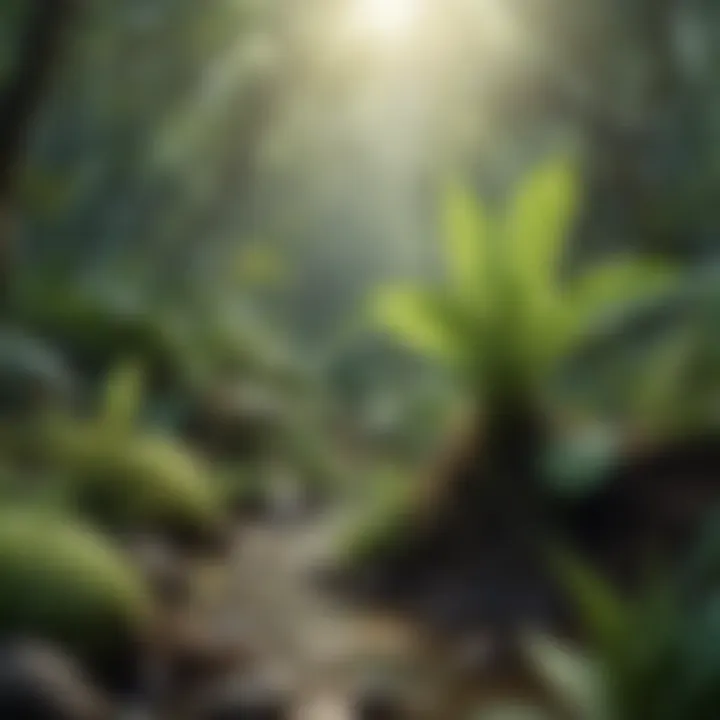
Meristematic Activity
Meristems are the regions in plants where active growth occurs, playing a crucial role in the development and regeneration of tissues. The apical meristem, located at the tips of roots and shoots, is particularly important as it gives rise to new cells that facilitate elongation and organ formation.
Within the meristem, cells remain undifferentiated and maintain their ability to divide. This is where a balance is maintained between cell division and differentiation. As cells divide in the meristematic zones, some leave this zone to undergo differentiation, developing specialized tissues. This intricate pattern of growth and differentiation allows for continuous development throughout the plant's life cycle, enabling it to adapt to its environment.
The signals that regulate meristematic activity are complex and multi-layered, involving both internal and external factors. Plant hormones, particularly cytokinins and gibberellins, are crucial for maintaining the balance of growth. While cytokinins promote the division of cells, gibberellins influence elongation and differentiation.
Furthermore, the activity of meristem cells can be affected by environmental factors such as light, temperature, and nutrient availability, which highlight the dynamic interplay between genetics and the environment in plant development.
"Understanding meristematic activity is not just about plants thrivingâit's about recognizing their adaptability in a world filled with change."
By comprehending these molecular mechanisms underpinning development, we gain insight into how plants not only grow but also respond to the world around them. This knowledge paves the way for innovations in agricultural practices and enhances our ability to safeguard plant species facing environmental challenges.
Plant Metabolism at the Molecular Level
Plant metabolism encompasses a variety of biochemical processes essential for plant growth, development, and survival. Understanding how plants harness energy and nutrients at the molecular level allows researchers to innovate in areas like agriculture and biotechnology. The study of plant metabolism unveils how plants convert sunlight into chemical energy and how they produce vital compounds that contribute to their resilience in the face of environmental stresses.
Metabolism includes two primary categories: anabolism (the building-up phase) and catabolism (the breaking-down phase). Each of these reactions plays a critical role not only in the sustenance of the plant itself but also in the overall health of the ecosystem. Why is this important? For one, it directly impacts crop yields and the ability to enhance food security amid a rapidly changing climate.
"Understanding plant metabolism is like unlocking the secrets of nature's largest factories, where sunlight is transformed into sustenance."
Photosynthesis and Its Molecular Basis
Photosynthesis stands as the cornerstone of plant metabolism, enabling plants to convert light energy into chemical energy. The process takes place in chloroplasts, which contain chlorophyllâa pigment responsible for capturing light. The overall reaction can be simplified as:
COâ + HâO + light energy â CâHââOâ + Oâ
This equation indicates that carbon dioxide and water, in the presence of light, are transformed into glucose and oxygen. But, what happens behind the scenes in this intricate dance?
The photosynthetic process can be divided into two main stages: the light-dependent reactions and light-independent reactions (Calvin cycle). During the light-dependent stage, sunlight is absorbed, driving the splitting of water molecules. This generates energy-rich molecules, ATP and NADPH, and releases oxygen as a byproduct.
Subsequently, in the Calvin cycle, ATP and NADPH are utilized to convert carbon dioxide into glucose. This conversion is not just a mere act of production; it's a multi-step process orchestrated by several enzymes and co-factors, emphasizing the complexity and efficiency of plant metabolism.
In the age of climate change, improving photosynthesis can lead to enhanced crop resilience and higher yields. Research is ongoing to identify ways to optimize this process through genetic engineering and metabolic manipulation.
Secondary Metabolite Production
Secondary metabolites are the unsung heroes of plant metabolism. Unlike primary metabolites such as glucose or amino acids, which are directly involved in growth and development, secondary metabolites serve critical ecological functions. These compounds can deter herbivores, attract pollinators, or even protect plants from pathogens.
Common classes of secondary metabolites include alkaloids, terpenoids, and flavonoids. For instance, alkaloids like caffeine and nicotine often have a toxic effect on herbivores, while terpenoids are used to produce essential oils that may serve as repellents. Besides their ecological roles, secondary metabolites also hold significant potential in pharmaceuticals and natural pesticides.
The production of secondary metabolites is tightly regulated by various pathways and signals within the plant. Stress factorsâsuch as drought, high temperatures, or attacks by pestsâcan lead to an upsurge in the synthesis of these compounds. This ability to respond to environmental changes makes secondary metabolites an interesting area for research, especially in the context of sustainable agriculture and health benefits.
Epigenetics in Plants
Epigenetics has emerged as a pivotal area of study within plant molecular biology. This branch of science explores how non-genetic factors can influence gene expression. Through mechanisms such as DNA methylation, histone modification, and RNA-associated silencing, plants can fine-tune their responses to ever-changing environmental conditions. The implications of epigenotic changes are profound, particularly as they relate to adaptation, development, and even yield in agricultural contexts.
Role of Epigenetic Modifications
The role of epigenetic modifications in plants cannot be overstated. These biochmical changes serve as a way for plants to record environmental signals and mediate gene expression accordingly. For instance, when plants are exposed to stressors like drought or pathogen attacks, they can alter their epigenetic landscape to activate or suppress certain genes that are crucial for stress response. This means that a plant can 'remember' past experiences and adjust its growth patterns in future seasons.
- DNA Methylation: This process involves the addition of a methyl group to the DNA molecule, usually silencing gene expression. In plants, this is critical for processes such as flowering time and resistance to disease.
- Histone Modification: Histones are proteins around which DNA is wrapped. Alterations to histones can lead to euchromatin or heterochromatin formation, influencing the accessibility of genes for transcription.
- Small RNA Molecules: These entities are essential for regulating gene expression and can degrade messenger RNA (mRNA) or inhibit its translation, hence impacting how genes are expressed under various conditions.
These modifications are not fixed but dynamic, allowing plants to swiftly respond to environmental changes. Understanding these mechanisms gives researchers new avenues to explore how to enhance crop resilience and productivity.
Phenotypic Variation and Adaptation
Phenotypic variation is fundamentally linked to epigenetics. This is because epigenetic changes can lead to observable differences in plant characteristics without altering the underlying DNA sequence. Phenotypic responses can vary greatly among individuals of the same genetic background when exposed to different environmental cues, a phenomenon often termed phenotypic plasticity.
"Epigenetics allows the imprint of environmental conditions to influence phenotypic outcomes across generations, without a change in the DNA sequence itself."
- Adaptation to Climate Change: As climate conditions shift, plants must adapt to survive. Epigenetic changes are vital in this process, enabling rapid adaptation. For example, studies have shown certain plant species can alter their flowering times in response to variations in temperature and humidity via epigenetic changes.
- Invasiveness and Biodiversity: Some plants can become invasive partly due to their ability to adapt quickly to new environments through epigenetic means. This rapid adaptability facilitates greater biodiversity, as these plants can fill ecological niches more adeptly than those relying solely on genetic alterations.
The exploration of epigenetics shines a light on not just how plants respond to their surroundings but also how such responses could be harnessed to improve agricultural practices. By understanding these intricate interactions and mechanisms, researchers and practitioners can develop crops that not only thrive but excel in fluctuating climates and conditions.
In summary, the study of epigenetics in plants offers a nuanced view into the myriad ways plants interact with their environment, providing essential insight into both fundamental biology and practical applications in agroecology.
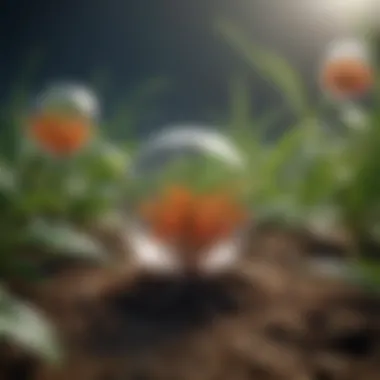
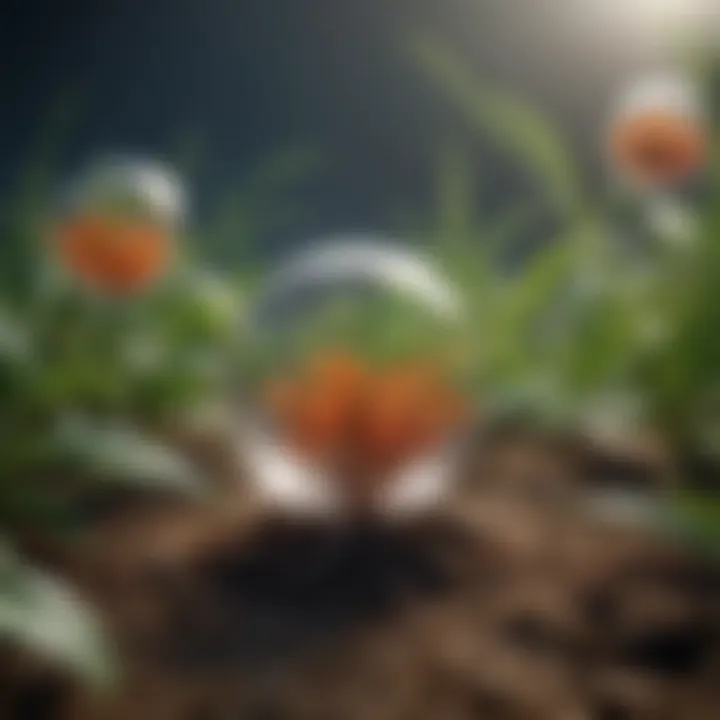
Interactions with Microorganisms
Interactions between plants and microorganisms are fundamental to understanding plant molecular biology. These interactions can broadly classifed into mutualistic (beneficial) relationships, commensal (neutral) relationships, and parasitic (harmful) interactions. The dynamics of these relationships can significantly influence plant health, growth, and ecological resilience.
Specifically, the study of these interactions sheds light on various aspects:
- Nutrient Exchange: Certain microorganisms assist in breaking down soil nutrients, making them more accessible for plant uptake.
- Disease Resistance: Some microorganisms can protect plants from pathogens, contributing to enhanced immune responses.
- Soil Health: The presence of beneficial microorganisms promotes soil structure and function, which is vital for sustainable agriculture.
Moreover, recent advancements in molecular techniques have allowed researchers to delve deeper into the genetic and biochemical pathways that underpin these interactions. Understanding these intricate relationships is more than just academicâit's crucial for improving agricultural practices and developing biotechnological solutions that can enhance sustainability.
Symbiotic Relationships
Symbiotic relationships, particularly those involving mycorrhizal fungi and nitrogen-fixing bacteria, represent a classic example of mutualism. Mycorrhizal fungi form extensive networks with plant roots, facilitating an efficient exchange of nutrients. In return, plants provide sugars and other organic compounds to the fungi. This partnership substantially enhances nutrient absorption, particularly phosphorus.
- Types of Mycorrhizae: There are mainly two types of mycorrhizal partnershipsâArbuscular Mycorrhiza (AM) and Ectomycorrhiza (EM). AM fungi penetrate plant roots more deeply while EM fungi typically envelop them.
- Nitrogen Fixation: Bacteria such as Rhizobium engage with leguminous plants to fix atmospheric nitrogen into a form accessible to plants. This interaction not only benefits the plants but also improves soil fertility for subsequent crops.
These symbiotic interactions drive the metabolic processes within plants and form a vital part of nutrient cycling within ecosystems.
Pathogen Responses
The ability of plants to recognize and respond to pathogens is a topic of immense interest in molecular plant biology. Plants have developed complex detection systems involving pattern recognition receptors (PRRs) that identify specific pathogen-associated molecular patterns (PAMPs). This recognition triggers defense responses aimed at curtailing pathogen spread.
- Defense Mechanisms: Upon detection, plants may initiate a variety of defense responses, including:
- Phytoalexin Production: These are antimicrobial compounds that accumulate at infection sites to inhibit pathogen growth.
- Cell Wall Reinforcement: Strengthening cell walls can prevent pathogen invasion and spread.
- Systemic Acquired Resistance (SAR): This is a long-lasting defense mechanism triggered by initial localized pathogen attack, allowing for a heightened state of readiness throughout the plant.
"Plants are not passive organisms in the face of threats; they actively engage in a biochemical warfare to safeguard their integrity."
In the broader context, studying plant-pathogen interactions not only enhances our understanding of plant biology but also aids in the development of disease-resistant crop varieties, which is crucial for food security in an era of changing climate conditions.
Biotechnological Applications in Plant Sciences
Biotechnology in plant sciences represents a critical intersection of biology and technology, heralding significant advancements in agricultural practices and ecological sustainability. The ability to manipulate plant genomes fosters innovations that address the growing demands for food security, resilience to climate change, and minimizing the environmental impact of farming. Techniques such as genetic engineering are not just enhancing what crops can offer but are also reshaping our understanding of plant biology itself. Various aspects of biotechnology illustrate its significance in the grander narrative of plant molecular biology.
Genetic Engineering Techniques
Genetic engineering is revolutionizing the way we approach crop improvement. By directly altering specific genes, scientists can enhance desirable traits such as drought resistance, nutrient efficiency, and pest tolerance. Techniques involved include:
- Agrobacterium-mediated transformation: Utilizing a naturally occurring bacterium to transfer genes into plant cells.
- Gene gun method: A physical method wherein DNA-coated particles are shot into plant tissues, resulting in transformation.
- Electroporation: Applying an electric field to increase DNA uptake by plant cells.
These approaches allow for precision in developing crops tailored to withstand environmental challenges. Consequently, genetic engineering plays a crucial role in developing crops that are not only high-yielding but also more adaptable to varying climatic conditions.
CRISPR and Its Impact
CRISPR has emerged as a game-changer in the realm of plant biotechnology. This powerful tool enables genome editing with unprecedented precision. By designing short RNA sequences, researchers can guide the CRISPR system to specific sites in the plant genome and create targeted alterations. Some notable impacts of CRISPR include:
- Precision breeding: Altering specific genes without inserting foreign DNA, thus maintaining the plant's natural gene pool.
- Enhanced traits: Developing crops with improved traits such as increased nutritional value, enhanced tolerance to pests, and resistance to diseases.
- Sustainable practices: Decreasing reliance on chemical pesticides and fertilizers through the development of resilient crops.
"CRISPR technology holds promise for not just improving food security but also paving the way for more resilient natural ecosystems."
As researchers delve deeper into the capabilities of CRISPR, the potential benefits extend beyond mere genetic modification. It poses questions about ethics, biodiversity, and the implications of such technologies in agriculture and beyond, compelling us to navigate these innovations with care. The fusion of biotechnology and plant sciences, particularly through genetic engineering and CRISPR applications, is shaping a future where agricultural practices can be both sustainable and productive.
Future Perspectives of Plant Molecular Biology
In looking forward to the future of plant molecular biology, it becomes evident that there are critical areas to consider not just for the scientific community, but for society as a whole. Emerging technologies and theories are revolutionizing our perception of how plants interact with their environment and how they can be manipulated for better yield, health, and sustainability. As the world grapples with issues like food security and climate change, the role of molecular biology in farming and conservation cannot be understated.
Sustainability and Crop Improvement
The quest for sustainability in agriculture is being greatly influenced by innovations in plant molecular biology. Modern techniques such as genetic modification and precise breeding programs are gaining traction. These methods allow scientists to introduce specific traits into plant genomesâtraits that can enhance resistance to pests, drought tolerance, and nutritional value. As a result, crops are being reengineered to thrive in varying environmental conditions, effectively pushing the boundaries of traditional agriculture.
There are several benefits arising from these advancements:
- Increased Yield: By optimizing genetic traits, itâs possible to create crop varieties that yield more per acre, addressing the pressing issue of food scarcity.
- Resource Efficiency: Molecular techniques can lead to plants that require fewer resources such as water and fertilizers, minimizing environmental impact.
- Nutritional Enhancements: By tweaking metabolic pathways, plants can be enhanced to provide more vitamins and minerals, essential in combating deficiencies prevalent in various populations.
However, these advancements do not come without considerations. Ethical questions surrounding genetically modified organisms (GMOs) need to be carefully addressed. Public perception and regulatory issues often hinder the acceptance of these technologies, highlighting the need for transparent discussions and educational outreach.
Impact of Climate Change on Molecular Processes
As climatic conditions shiftâbe it through rising temperatures, changing precipitation patterns, or increased carbon dioxide levelsâplants face a multitude of stressors that can disrupt their molecular processes. Understanding how molecular biology can adapt or mitigate these effects is imperative for future plant health and yield.
- Altered Photosynthesis: Changes in environmental conditions can affect the efficiency of photosynthesis, the process by which plants convert light into energy. Adjustments at the molecular level may be necessary to enhance photosynthetic pathways to cope with these alterations.
- Stress Responses: The interaction between stress-related genes and proteins can determine plant survival. Research is being directed to uncover how plants sense stress and which signaling pathways get activated, leading to improved resilience strategies.
- Shifts in Plant-Soil Microbe Interactions: Climate change can also affect the dynamics between plants and symbiotic microorganisms in the soil, which are essential for nutrient uptake. Understanding these complex interactions at a molecular level is critical for developing sustainable agricultural practices.
"The future will be defined by how effectively we utilize our knowledge of molecular biology to meet the challenges of climate change and agricultural demand."