Introduction to DNA: Structure, Function, and Implications
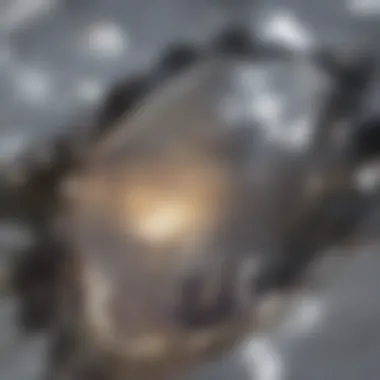
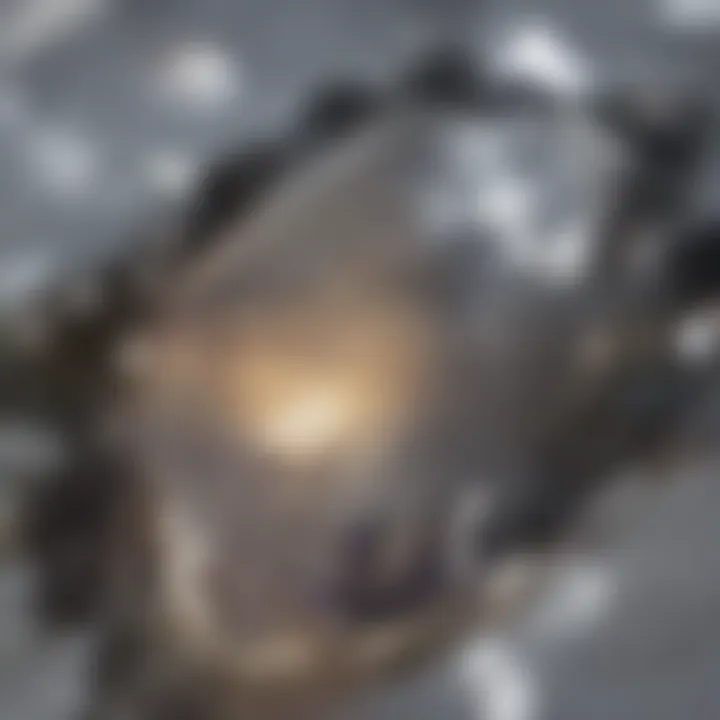
Intro
DNA, or deoxyribonucleic acid, serves as the blueprint for all living organisms. Understanding DNA is essential not only for biology but also for many fields such as medicine, genetics, and biotechnology. This article explores the fundamental aspects of DNA, including its remarkable structure, its critical functions in living cells, and the far-reaching implications that advancements in genetic research bring to science and society. The journey of DNA study has altered our comprehension of life, revealing intricate details that govern hereditary traits and biological processes.
Key Findings
- Structure of DNA: DNA consists of two intertwined strands forming a double helix. Each strand is made up of nucleotide units, which comprise a sugar, a phosphate group, and one of four nitrogenous bases: adenine, thymine, cytosine, or guanine. The specific pairing of these bases is foundational to genetic coding.
- Functional Mechanisms: DNA functions primarily in storing and transferring genetic information. This information is vital for cell function, replication, and repair. Protein synthesis is one critical process guided by DNA, where the information is transcribed and translated into proteins that carry out various functions in the body.
- Historical Context: The discovery of DNA occurred in a multifaceted historical landscape, with contributions from key figures in science. Names like Watson, Crick, and Franklin echo through the annals of molecular biology for their roles in revealing the structure and function of DNA.
Key findings underscore that not only is DNA essential for life, but its understanding is also crucial for scientific and medical advancements.
Implications of the Research
- Real-World Applications: The implications of DNA research range from medical advancements, such as gene therapy, to biotechnology applications, such as genetically modified organisms. DNA sequencing technology has paved the way for personalized medicine, where healthcare can be tailored to an individual's genetic profile.
- Future Research Directions: As genetics research evolves, it raises crucial ethical questions surrounding genetic modification, cloning, and privacy. It is vital to navigate these issues carefully, ensuring that technology is used responsibly and effectively.
Understanding DNA
Understanding DNA is crucial for grasping the fundamental principles of molecular biology. DNA is at the core of genetic information in all living organisms. Its structure and function offer insight into how traits are inherited and how species evolve. Recognizing the importance of DNA aids in fields such as genetics, medicine, and biotechnology.
The benefits of understanding DNA are numerous. It enhances our grasp of hereditary diseases, supports advances in genetic engineering, and plays a significant role in forensic science. Furthermore, it provides a basis for developments in personalized medicine, allowing for treatments tailored to an individual's genetic profile.
Considering these factors, understanding DNA goes beyond mere academic interest; it molds future medical practices and scientific discoveries that can impact human life significantly.
Definition of DNA
DNA, or deoxyribonucleic acid, is a molecule that carries the genetic instructions used in the growth, development, functioning, and reproduction of all known living organisms. Structurally, DNA consists of two long strands forming a double helix. These strands are composed of smaller units called nucleotides. Each nucleotide includes a sugar molecule (deoxyribose), a phosphate group, and one of four nitrogenous bases: adenine, thymine, cytosine, or guanine.
It serves as a blueprint for the synthesis of proteins, which execute various functions essential for life.
Historical Context
The quest to understand DNA dates back to the 19th century. In 1869, Swiss chemist Friedrich Miescher first isolated nucleic acids, which he called "nuclein." This was the starting point of DNA research. The pivotal moment came in 1953 when James Watson and Francis Crick proposed the double helix structure of DNA. Their model revealed how genetic information could be stored, replicated, and transmitted.
This discovery laid the groundwork for molecular biology, changing our understanding of genetics forever. It led to significant developments including the Human Genome Project, which aimed to map all human genes. The history of DNA is not merely about scientific advancement; it reflects societal shifts that influence health, ethics, and technology.
Only by grasping the foundational history of DNA can we appreciate its intricate role in both science and society.
Structure of DNA
The structure of DNA is fundamental to understanding its role in biological systems. The arrangement of this molecule allows it to store genetic information in an organized manner, ensuring that the necessary information for life can be replicated and transmitted between generations. This section will focus on the two critical elements of DNA structure: the double helix configuration and nucleotide composition. These aspects are not just structural foundations but also crucial to DNA's functions.
Double Helix Configuration
The iconic double helix structure of DNA was discovered by James Watson and Francis Crick in 1953. This configuration is significant for several reasons. Firstly, it provides stability to the molecule. The two strands are held together by hydrogen bonds between complementary nitrogenous bases, making it strong yet flexible. This design supports the accurate replication of genetic material.
Moreover, the twisting of the double helix allows for efficient packing of DNA within cells. This compactness is crucial, given that the length of DNA in a single human cell can reach up to two meters. The double helix not only defines the structure but also influences the function of DNA through its ability to unwind and separate for replication and transcription.
Nucleotide Composition
The composition of nucleotides is another essential element in understanding DNA structure. Each nucleotide consists of three components: deoxyribose sugar, phosphate backbone, and nitrogenous bases.
Deoxyribose
Deoxyribose is the sugar component of DNA nucleotides. This five-carbon sugar is unique due to the absence of one oxygen atom compared to ribose, the sugar found in RNA. The lack of this oxygen makes deoxyribose more chemically stable, which is essential in preserving the integrity of genetic information over time.
One key characteristic of deoxyribose is its role as a building block for nucleotides. Each deoxyribose molecule bonds with a phosphate group and one of four nitrogenous bases, forming the structural foundation of DNA. Its stability allows DNA to serve as a long-term storage medium for genetic information, which is advantageous for processes like replication and transcription.
Phosphate Backbone
The phosphate backbone refers to the chain of phosphate groups that links nucleotides together in a DNA strand. This backbone provides structural integrity to the molecule. The repeating sugar-phosphate units form the sides of the DNA ladder, while the nitrogenous bases form the rungs.
A significant aspect of the phosphate backbone is its role in the overall polarity of DNA. Each strand of DNA has a direction, often referred to as 5' to 3'. This polarity is critical during the DNA replication process, as enzymes work in a specific direction when synthesizing new strands.
Nitrogenous Bases
The nitrogenous bases are the information carriers of DNA. There are four types of nitrogenous bases in DNA: adenine, thymine, cytosine, and guanine. The specific pairing of these bases—adenine with thymine, and cytosine with guanine—forms the characteristic rungs of the double helix.
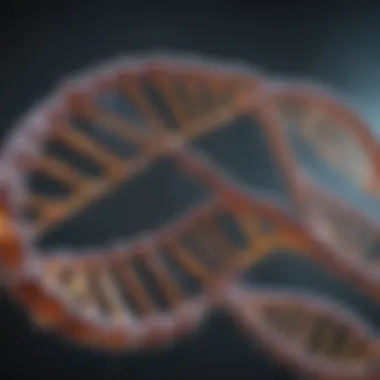
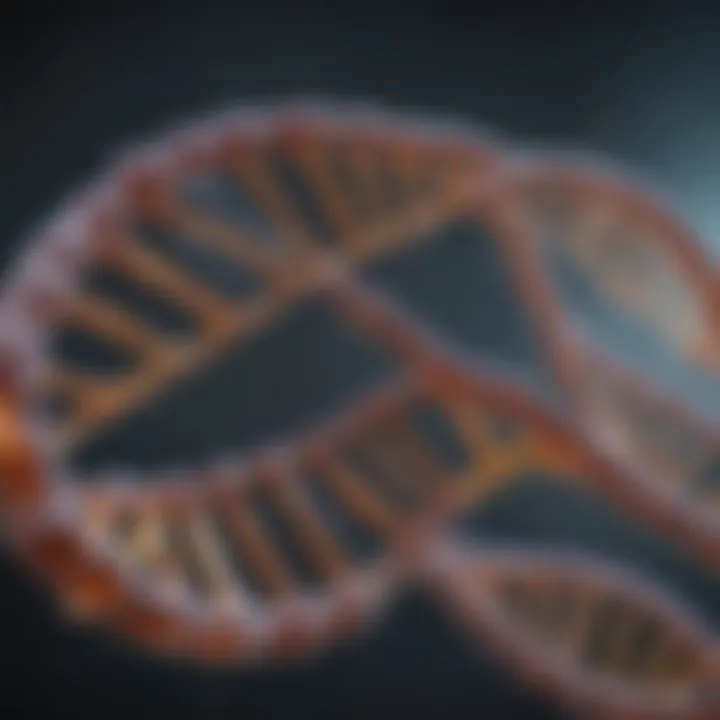
The unique feature of nitrogenous bases lies in the genetic code they establish. The sequence of these bases encodes the information necessary for protein synthesis and other cellular functions. Additionally, the complementary base pairing ensures that genetic information can be accurately copied during replication. This fidelity is crucial for maintaining the stability of genetic characteristics across generations.
"The structure of DNA is closely linked to its function, bearing a direct influence on the mechanisms of genetic inheritance and expression."
Function of DNA
Understanding the function of DNA is critical for comprehending how biological systems operate. DNA serves not just as a static blueprint, but as a dynamic and functional entity in cellular activities. It plays a vital role in the storage and transmission of genetic information as well as in cellular processes like replication and protein synthesis.
Genetic Information Storage
DNA's primary function is genetic information storage. It holds the instructions needed for growth, development, and the maintenance of all living organisms. The sequence of nucleotides in DNA encodes the necessary information that dictates cellular function. Each segment of DNA, known as a gene, corresponds to specific traits. The storage capacity of DNA is immense, allowing it to hold enough information to code for thousands of proteins within just a few millimeters of material.
Replication Process
Replication is a fundamental mechanism that allows DNA to duplicate itself, ensuring that genetic information is passed on during cell division.
Enzymes Involved
A crucial aspect of the replication process is the involvement of various enzymes. The key enzyme is DNA polymerase, which adds nucleotides to form new strands based on the templates provided by the original DNA strands. This enzyme is vital for ensuring accurate copying of genetic material. Its speed and ability to proofread newly synthesized DNA help maintain the integrity of genetic information, making it a beneficial factor in cellular function. However, errors can still occur, leading to mutations.
Mechanisms of Replication
The mechanisms of replication include various steps that work in concert to ensure successful duplication. The process initiates at specific locations on the DNA, known as origins of replication. DNA unwinds, creating replication forks where enzymes can work. Nucleotides are then paired according to base-pairing rules, and the backbone is assembled. This coordinated action of multiple enzymes is essential for efficient replication. It is a strong choice for maintaining fidelity in genetic copying, though it is also subject to certain limitations, such as the handling of complex genomes.
Protein Synthesis
Another critical function of DNA is in protein synthesis, which encompasses two main processes: transcription and translation. In this way, DNA is responsible for producing the proteins that carry out virtually every function in a cell.
Transcription
Transcription is the first step of protein synthesis where a specific segment of DNA is transcribed into messenger RNA (mRNA). The enzyme RNA polymerase facilitates this process, adding RNA nucleotides complementary to the DNA template strand. This process is essential as it converts DNA's static information into a dynamic form that can be translated into a protein. The mRNA then carries this information to the ribosomes for translation. While transcription is a generally accurate process, errors can occur, which may affect protein functionality.
Translation
Translation is the process wherein ribosomes read the sequence carried by mRNA and synthesize proteins accordingly. It involves several components including transfer RNA (tRNA), which brings amino acids to the ribosome, and ribosomal RNA (rRNA), which plays a structural and catalytic role in protein synthesis. The efficiency of translation is crucial for cellular function, as proteins formed in this process perform numerous vital roles within the organism. Inaccuracies during translation can lead to dysfunctional proteins, impacting various biological processes.
Overall, the functions of DNA are central to life, linking genetic information storage, replication, and the synthesis of proteins, without which organisms cannot function effectively.
Genetic Variation
Genetic variation is critical in understanding the diversity of life and the evolution of organisms. It refers to the differences in genetic makeup among individuals within a population. This variation plays a central role in adaptability, evolution, and the mechanisms of inheritance. Examining genetic variation can inform scientists about disease resistance, behavioral traits, and other attributes critical for survival and reproduction.
Mutations
Mutations are alterations in the DNA sequence that can lead to changes in phenotype. They may occur spontaneously or due to environmental factors such as radiation or chemicals. Understanding mutations gives insight into the sources of genetic variation; without them, evolution would not proceed as it does.
Types of Mutations
The types of mutations can be classified into several categories:
- Point mutations: A single nucleotide is changed, inserted, or deleted.
- Insertions and deletions (indels): One or more nucleotides are added or removed.
- Frameshift mutations: Caused by indels that alter the reading frame of the genetic code.
Each type has unique implications. Point mutations have a subtle effect, while frameshift mutations can have dramatic consequences on protein functionality. Their examination helps illustrate the fundamental mechanisms of evolution and disease. Therefore, studying these types is a beneficial choice for understanding how genetic variation works and influences traits.
Effects of Mutations
Mutations can lead to various effects, ranging from benign to harmful. Some common effects are:
- Neutral mutations: Have no effect on the organism's fitness.
- Beneficial mutations: Enhance fitness, leading to adaptive traits.
- Deleterious mutations: Decrease fitness, potentially leading to health issues or death.
The impact of mutations must be assessed carefully. For instance, beneficial mutations could improve resistance to diseases, while deleterious mutations might cause genetic disorders. Examining these effects allows researchers to understand the interplay between mutation, natural selection, and survival.
Genetic Inheritance
Genetic inheritance refers to how traits and genetic information are passed from parents to offspring. It lays the groundwork for understanding population dynamics, genetics, and the means by which traits persist or evolve.
Mendelian Inheritance
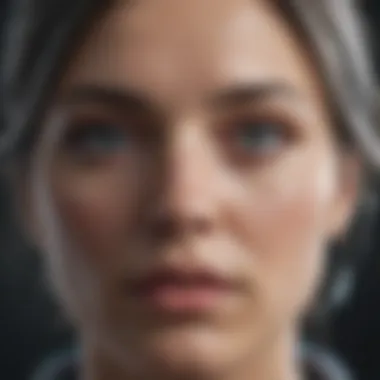
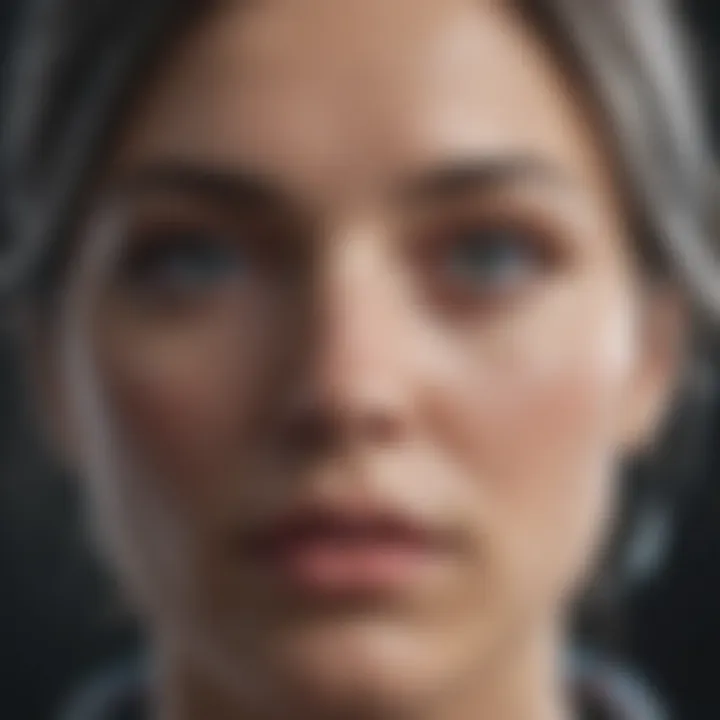
Mendelian inheritance describes patterns of inheritance first established by Gregor Mendel. It revolves around concepts like dominant and recessive alleles.
Key principles include:
- Law of Segregation: Alleles segregate independently during gamete formation.
- Law of Independent Assortment: Genes for different traits segregate independently.
This framework is crucial for studying genetic variations. Mendelian inheritance provides valuable insights into traits that exhibit clear inheritance patterns, such as those following simple dominant/recessive relationships. This is relevant for predicting genetic outcomes in breeding experiments and genetic counseling.
Complex Traits
Complex traits are influenced by multiple genes as well as environmental factors. Examples include height, weight, and susceptibility to certain diseases.
Characteristics of complex traits:
- Polygenic: Involves multiple genes and interactions among them.
- Environmental Influence: Factors like nutrition can affect the expression of these traits.
Understanding complex traits is significant as it helps illuminate how genetic variation operates beyond Mendelian principles. Recognizing the role of multiple genetic contributions can lead to improved disease risk assessments and targeted interventions.
Technology and DNA
In the modern landscape of genetic research, technology plays a pivotal role in enhancing our understanding of DNA. Advancements in various techniques have facilitated groundbreaking discoveries, revolutionizing the fields of genetics, medicine, and biotechnology. This section delves into notable technologies, their significance, and their implications for future research and applications.
DNA Sequencing Techniques
Sanger Sequencing
Sanger sequencing, developed by Frederick Sanger in the 1970s, revolutionized genetic analysis. The method allows for the precise determination of nucleotide sequences in DNA. It utilizes labeled dideoxynucleotides, which terminate DNA strand elongation during replication, producing fragments of varying lengths. A major characteristic of Sanger sequencing is its accuracy. This makes it a preferred choice for smaller-scale projects, such as sequencing individual genes or verifying sequences obtained from other techniques.
Advantages of Sanger sequencing include:
- High Accuracy: It provides reliable results due to the robust methodology.
- Established Protocols: Many labs have standardized procedures for its use.
However, its main disadvantage lies in throughput. Sanger sequencing is less efficient for large-scale projects, making it less suitable for denser genomic regions.
Next-Generation Sequencing
Next-generation sequencing (NGS) marks a significant leap from Sanger sequencing. It encompasses multiple high-throughput techniques that allow for simultaneous sequencing of millions of DNA strands. NGS is characterized by its speed and scalability. It quickly generates large volumes of data, facilitating comprehensive genomic studies.
One unique feature of NGS is its ability to sequence entire genomes in a fraction of the time it takes using traditional methods. This technology is particularly beneficial for:
- Whole Genome Sequencing: Enables entire genomes to be sequenced efficiently.
- Mutational Analysis: NGS can identify numerous mutations across various samples.
Despite its advantages, NGS comes with challenges, including:
- Data Management: The immense amount of data generated requires sophisticated storage and analysis tools.
- Technical Expertise: It demands skilled personnel for data interpretation.
CRISPR and Gene Editing
Mechanism of CRISPR
CRISPR technology provides a powerful tool for precise gene editing. Originally a bacterial defense mechanism, it was adapted for targeted genome modifications. The mechanism consists of guide RNA that directs the Cas9 enzyme to specific DNA sequences to induce double-strand breaks. The cell then attempts to repair these breaks, often leading to gene disruption or modification.
The key characteristic of CRISPR is its target specificity, which allows for precise edits at predetermined locations in the genome. This makes it a favored option for researchers aiming to study gene function or develop therapeutic strategies.
Advantages of CRISPR include:
- Versatility: It can modify multiple genes simultaneously.
- Cost-Effectiveness: The procedure is more affordable than previous gene-editing methods.
However, CRISPR also raises concerns, such as:
- Off-Target Effects: Unintended edits may occur, resulting in unintended consequences.
Applications in Medicine
The applications of CRISPR technology in medicine are numerous. It opens avenues for treating genetic disorders by correcting mutations at their source. Furthermore, it assists in developing targeted therapies for cancers and infectious diseases. The ability to engineer immune cells enhances the efficacy of treatments for various diseases.
A significant feature of CRISPR's medical application is its ability to create tailored treatments for patients based on their genetic makeup. It allows for:
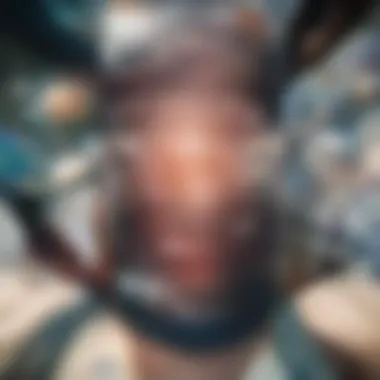
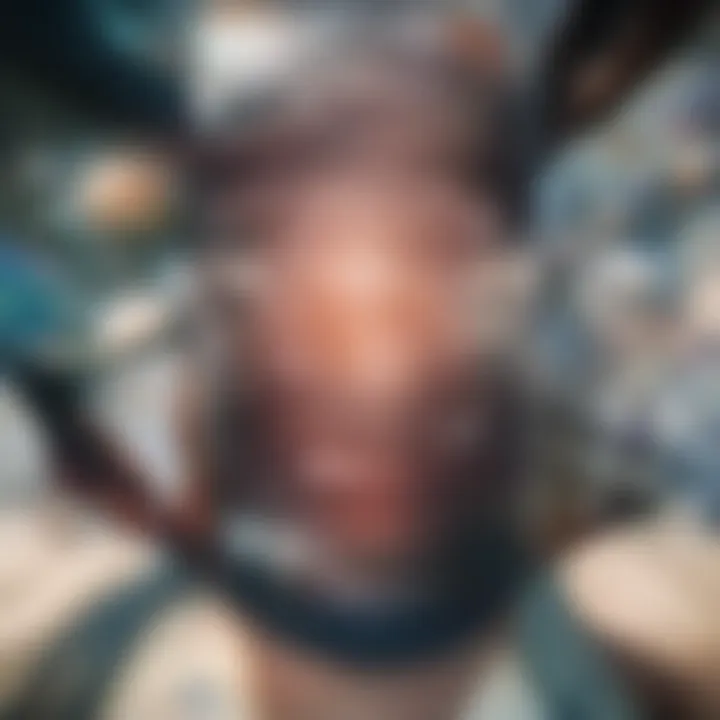
- Personalized Medicine: Treatments can be designed to fit individual patients genetic profiles.
- Disease Eradication: Potential to eliminate genetic disorders at their root.
Nonetheless, challenges exist, including:
- Ethical Implications: Editing the human germline raises profound moral questions.
"The potential impact of CRISPR technology in medicine is profound, yet it comes with responsibilities that the scientific community must carefully navigate."
Ethical Considerations
The intersection of DNA research and ethics is increasingly crucial as advancements in genetic technology reshape our understanding of biology. Ethical considerations inform policy and practice, shaping how genetic information is used and who benefits from these innovations. This section addresses two significant aspects of ethical debate within DNA research: the privacy of genetic data and the ethics surrounding germline editing.
Privacy in Genetic Data
In an age where genetic testing has become more accessible, the question of privacy in genetic data is paramount. Genetic information reveals vast details not only about an individual but also about family members, opening up concerns regarding consent, autonomy, and the misuse of sensitive information. The potential for data breaches raises alarms over unauthorized access to personal genetic data, potentially leading to discrimination in various sectors, including insurance and employment.
Researchers and companies that handle genetic data must establish clear guidelines and protocols to protect individuals' privacy rights. Individuals should have the right to control how their genetic information is shared and utilized. This involves informed consent processes that fully explain risks and benefits, ensuring that participants understand the implications of their decisions.
"Balancing innovation with privacy rights is vital to maintaining public trust in genetic research."
As the landscape of genetic research evolves, ethical frameworks must adapt. Ensuring robust protection of genetic privacy is essential for fostering public participation in genetic research while mitigating risks of exploitation and harm.
Germline Editing Ethics
Germline editing presents significant ethical challenges, given its potential to alter the hereditary information passed down through generations. Technologies like CRISPR have made it possible to edit genes in embryos, raising profound moral questions. The potential for misuse, combined with the uncertainty of long-term effects, necessitates careful deliberation about when and how this technology should be applied.
Opponents of germline editing often express concerns about the unforeseen consequences on human evolution and biodiversity. Issues related to "designer babies" — selecting traits such as intelligence or physical appearance — confront our understanding of social justice.
Furthermore, ethical voices advocate for rigorous assessments of potential risks associated with germline therapies. Assessing the health implications for future generations should require thorough scientific scrutiny and public discourse.
As we explore the frontiers of genetic engineering, establishing a clear ethical framework for germline editing is paramount. This will guide responsible research practices while addressing the public’s moral apprehensions regarding these advancements.
Future of DNA Research
The future of DNA research holds significant promise for advancements in medicine, biotechnology, and our understanding of genetics. As technologies evolve, the capacity to manipulate and understand DNA continues to grow. This section discusses the potential applications of DNA research, as well as the challenges that may arise in its journey towards meaningful implementation.
Potential Applications
Personalized Medicine
Personalized medicine represents a cutting-edge approach where medical treatment is customized to the individual based on their genetic makeup. This concept is gaining traction as research uncovers the link between genetics and health outcomes.
A key characteristic of personalized medicine is its ability to tailor interventions for specific genetic profiles. This approach not only aims to improve effectiveness but also to minimize side effects. For instance, cancer therapies can be designed based on the genetic mutations found within a patient’s tumor, making treatments more effective.
The unique feature of this method lies in its reliance on genomic data, which enables healthcare providers to predict responses to medications. Benefits of personalized medicine include enhanced therapeutics and improved patient outcomes. However, potential disadvantages include the high costs of treatments and ethical concerns related to access and privacy.
Gene Therapy
Gene therapy aims to treat or prevent diseases by directly altering the genes inside a patient's cells. This innovative technique has the potential to address genetic disorders at their source.
The key characteristic of gene therapy is its focus on correcting or replacing faulty genes. This method can provide long-lasting solutions to otherwise chronic conditions. One notable aspect is its ability to potentially cure diseases, a significant consideration for areas like cystic fibrosis or hemophilia.
Gene therapy’s unique feature is its application via various vectors, such as viruses. While this offers a mechanism for delivering therapeutic genes, there are risks involved, including immune reactions and the possibility of unintended consequences. Therefore, despite its promise, gene therapy presents both opportunities and challenges that merit careful consideration.
Challenges Ahead
Technological Barriers
Technological barriers encompass limitations in current tools and methodologies that hinder research and application in DNA studies. Advancements in sequencing and editing technologies are necessary to fully realize the benefits of DNA research.
One of the key characteristics of these barriers is the complexity of biological systems. Understanding gene interactions and expressions is not straightforward. The rapidly evolving field requires continual adaptation and investment in research. Without addressing technological gaps, the integration of findings into clinical practice may remain slow.
Unforeseen difficulties, such as data management and accuracy in gene editing, pose further concerns. These limitations can restrict the pace at which new applications are brought to market, thereby impacting potential public health benefits.
Public Perception
Public perception plays a crucial role in how DNA research progresses. The way society views genetic engineering, privacy, and safety shapes the landscape for future research.
A significant characteristic of public perception is its impact on policy and regulation. As advancements occur, public opinion can lead to policies that either promote or hinder research initiatives. Education and transparency are critical here. Misunderstandings can create fear, influencing regulatory frameworks in ways that may limit innovation.
The unique feature of public perception is its variability; different communities may have differing viewpoints based on cultural or ethical considerations. Thus, engaging the public and addressing concerns is vital for the successful implementation of DNA advancements.
"To navigate the future of DNA research, we must balance innovation with ethical considerations, ensuring inclusivity and understanding in society."